LII:Basic Physics of Nuclear Medicine/Dual-Energy Absorptiometry
Introduction
[edit | edit source]This is a developing chapter of a wikibook entitled Basic Physics of Nuclear Medicine.
Dual-energy radiography is an imaging technique which can be used to eliminate bone information in an radiograph, so that an image displaying tissues only can be displayed. Alternatively, the technique can be used to generate the reverse effect where tissue information is eliminated and an image displaying bone only is generated. This latter option ideally allows indicators of bone density to be assessed. A theoretical background to this technique will first be developed below with the discussion leading towards Dual-Energy X-Ray Absorptiometry (DEXA).
Dual-Energy Radiography
[edit | edit source]Dual-energy imaging is based on exploiting the difference in the attenuation of tissue and bone at different X- ray energies – see the following figure:

It generally involves acquiring images at two X-ray energies and processing these images to suppress either the bone or the tissue information. A simple mathematical model assumes that monoenergetic radiation is used and no scattered radiation is detected, so that the transmitted radiation intensity through a region of bone and tissue, acquired at a low X-ray energy and following logarithmic transformation, is given by:
where:
- μtlo is the linear attenuation coefficient of tissue at the low X-ray energy;
- xt is the tissue thickness;
- μblo is the linear attenuation coefficient of bone at the low X-ray energy; and
- xb is the bone thickness.
Similarly, the transmitted radiation intensity for the same region of an image acquired at a higher X-ray energy is given by:
where:
- μthi is the linear attenuation coefficient of tissue at the higher X-ray energy; and
- μbhi is the linear attenuation coefficient of bone at the higher X-ray energy.
When these images are multiplied by separate weighting factors, klo and khi, and the result combined to form a composite image, the output image is given by:
Therefore:
which indicates that tissue cancellation can be achieved by setting the coefficient of xt equal to zero, i.e.
Thus,
and
which indicates that tissue can be eliminated from the composite image when the ratio of weighting factors in equation (1) above is chosen to equal the negative of the ratio of the attenuation coefficients of tissue at the two X-ray energies. A similar approach can be used to cause bone cancellation by setting the coefficient of xb in equation (1) to zero.
This form of image data processing is illustrated in the following figure:

A chest radiograph acquired at 56 kVp is shown in the top left panel of the figure. This is referred to as a low energy image. In the top right panel is a radiograph of the same patient's chest acquired at a high energy – 120 kVp, with 1 mm copper filtration. Results of the dual-energy processing are shown on the bottom row. The bone-subtracted image is shown in the bottom left panel and the tissue-subtracted image in the bottom right panel. Notice that the tissue-subtracted image demonstrates that the lesion in the patient's left lung is a calcified nodule, since it doesn't appear in the bone-subtracted image.
Radiographic Image Receptors
[edit | edit source]There are at least three new technologies used in radiography which offer numerous advantages over the traditional screen/film image receptors. An overview of these technologies is provided below to support our treatment of dual-energy imaging – and also our consideration of the registration and fusion of planar images in another chapter of this wikibook.
- Computed Radiography (CR)
- This method of recording a projection radiograph is based on exploiting the photostimulable luminescent properties of barium fluorohalide compounds. The phosphor is layered on an imaging plate which when mounted in a cassette is used in place of the film/screen cassette used traditionally in radiography. The imaging plate is sometimes referred to as a storage phosphor because it stores a latent image following the radiation exposure which can be scanned afterwards using a readout device – as illustrated in the following figure:

- The figure shows the radiation exposure in the top left where the cassette is placed anterior to the patient to record the projected image. The cassette is then placed in a readout device (follow the blue downward arrow!), where it is scanned by a laser beam to digitize the latent image. The cassette can then be prepared for re-use by exposing it to intense incandescent light to erase any residual latent image information (top right in the figure above).
- The readout stage is shown in more detail in our next figure:

- The figure illustrates one mechanism used to scan the imaging plate where a narrow laser beam strikes a rotating mirror causing it to scan a single line across the plate. The plate is then moved so that the scanning laser beam can read the next line of the latent image information.
- The latent image is formed through radiation absorption processes in the photostimulable phosphor where electrons are knocked into higher energy states where they remain until they're later stimulated to return to their ground states using the red laser beam – a process similar to thermoluminescence where light is used instead of heat. The electrons emit blue light – called a photostimulable luminescence (PSL) – as they return to their ground states with the amount of light being proprotional to the radiation exposure.
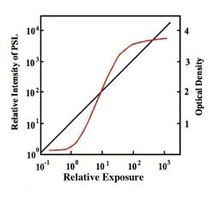
- The emitted light is guided by a light guide, as shown in the figure above, so that its intensity can be measured using a photomultiplier tube (PMT). The output of the PMT is digitized using an analogue-to-digital converter (ADC) before computer processing is applied.
- One major advantage of computed radiography (CR) over traditional methods is its excellent linearity and dynamic range, as illustrated by the graph. The vertical axis on the left of the graph refers to the response of a CR plate, while the one on the right refers to a film/screen system. Its seen that the film/screen response (red curve) contains regions which can generate under- and over-exposures of the radiographic film. The linear region between these two is ideally used for recording a patient's image. Notice that the range of exposures equivalent to this linear region is substantially smaller than that provided by CR technology, i.e. its got a smaller dynamic range (also called exposure latitude in photography).
- The important point to appreciate here is that CR images present data which is a superior record of the radiation exposure pattern incident on the cassette. They do not contain regions of over- and/or under-exposure, as is common is film/screen radiography. An improved ability to discriminate within regions of high (or indeed low) transmission results, so that, for example, a renal calculus can be readily depicted within a contrast filled renal pelvis in an IVP. Note that the dynamic range of CR is rather broad and that contrast enhancement techniques are typically applied in a post-processing mode to window through the image data so that particular features of interest can be discerned. Example CR images are shown below:

- This linear, wide dynamic range characteristic is also shared by digital radiographic image receptors.
- Digital Radiography (DR)
- Considerable research has been conducted in recent years into the development of flat panel image receptors for digital radiography. This research has extended from the development of active-matrix liquid-crystal flat panel displays (AMLCDs) for application in, for example, portable computers. The underlying technology of AMLCDs is a large area integrated circuit called an active matrix array which consists of many millions of identical semiconductor elements deposited on a substrate material. An intensifying screen or a photoconductor coupled to such an active matrix array forms the basis of flat panel X-ray image receptors.
- Such a receptor is illustrated in the following figure, where the active matrix array and associated electronic circuitry is mounted in a device which replaces the X-ray cassette in screen-film radiography:

- Array sizes of up to 43 cm x 43 cm have been constructed with more than 9 million pixels (pixel size ~150 μm). Operation of the array is controlled by a digital image processor which also stores and displays the resultant images.
- The operation is illustrated in more detail in the right panel of the above figure. Each pixel of the array has a switch (typically made from a thin film transistor) which is connected to switching control circuitry in a manner which allows all switches in a row of the array to be operated simultaneously. The output from each pixel is connected in columns with individual pre-amplifiers.
- All switches are kept in the off position during the X-ray exposure. Following the exposure, the switches in the first row are turned on and the signal from each pixel is amplified by the pre-amplifiers, digitised in an analogue-to-digital converter (ADC) and stored in the image memory of the digital image processor. These switches are then turned off and the switches in the second row are turned on to acquire signals from the second row of pixels. This process is repeated for the whole array so that an image is acquired in a sequential, line-by-line manner.
- Research developments have resulted in two distinct types of digital receptor:
- Indirect Image Receptors
- Indirect receptors are based on coupling an fluorescent screen to the active matrix array. Phosphors such as Gd2O2S:Tb and CsI:Tl have been used, and the light produced following X-ray interaction is detected by an array of pixels consisting of photodetectors – see the following figure:

- Each photodetector generates an electric charge that is proportional to the amount of light striking it, and this charge is stored until it is read-out by the switching control circuitry. The detection process is referred to as indirect since the detected X-rays are first converted to light, which is subsequently converted to electric charge.
- Direct Image Receptors
- Direct receptors are based on coupling a photoconductor to the active matrix array. Photoconductors such as amorphous selenium (a-Se) have been used, and the electric charge produced following X-ray interaction is detected by an array of pixels each consisting of an electrode and a capacitor – see the following figure:

- This charge is stored in each capacitor until it is read-out by the electronic switching circuitry. The photoconductor requires a voltage of the order of 5,000 V to be applied, using a surface electrode, so that the charge produced can be attracted to the pixel electrodes. Other photoconductors under investigation for this application include PbI2, PbO, TlBr and CdTe.
- The photoconductive properties of amorphous selenium (a-Se) have been known for many years and have been widely applied in photocopier, facsimile and laser printer devices. This direct imaging technique has evolved from xeroradiography, which was a popular medical imaging technique many years ago mainly for soft tissue imaging. Here, X-ray absorption in the photoconductor results in changes in the distribution of the electric charge reflecting local X-ray absorption, i.e. a latent image is recorded in terms of the distribution of electric charge on the surface of an a-Se plate. Methods of rendering the latent image visible include:
- spraying charged toner particles onto the plate and transferring this image to paper;
- scanning the plate with electric charge transducers (e.g. electrometers) and digitising the output for storage in computer memory.
- The toner approach was that adopted in the original xeroradiography systems and is no longer in widespread clinical use, mainly due to developments in film-screen technology. The scanning electrometer approach has been applied to the clinical imaging of chests with the Philips Thoravision system. This involves use of an a-Se layer deposited on a cylinder. The latent image is recorded on the surface of the layer and the drum is subsequently rotated past a linear array of tiny electrometers. In comparison, the direct image receptors use an a-Se layer in intimate contact with a two-dimensional array of pixel-sized electrodes, as we have seen above.
- The photoconductive properties of amorphous selenium (a-Se) have been known for many years and have been widely applied in photocopier, facsimile and laser printer devices. This direct imaging technique has evolved from xeroradiography, which was a popular medical imaging technique many years ago mainly for soft tissue imaging. Here, X-ray absorption in the photoconductor results in changes in the distribution of the electric charge reflecting local X-ray absorption, i.e. a latent image is recorded in terms of the distribution of electric charge on the surface of an a-Se plate. Methods of rendering the latent image visible include:
- Important design features of flat panel image receptors include the pixel size and the fill factor. Pixel size undoubtedly affects spatial resolution and sizes of the order of 100 – 200 μm are typical. The fill factor is the percentage of a pixel area which is sensitive to the image signal – be it electric charge or light photons. It is never 100%, given the need to accommodate conductors (~10 μm wide) which input switching signals and which output image signals, as well as the thin film transistor in each pixel.
- Digital image processing techniques are typically applied to acquired images before they are displayed. These include logarithmic transformation, to correct for exponential X-ray attenuation, and contrast enhancement, to optimise the displayed gray values. Other digital processing techniques are also necessary to overcome limitations associated with the manufacturing process for individual image receptors. These include flat field (i.e. uniformity) corrections, to overcome spatial sensitivity variations, and median filtering, to remove the effects of defective pixels.
- Advantages of the indirect and direct image receptors purport to include a large, linear dynamic range, similar to photostimulable phosphors, and superior modulation transfer function (MTF) and detective quantum efficiency (DQE) compared with both film-screen and photostimulable phosphor systems. Such receptors are currently (i.e. in 2006) being introduced by major medical imaging companies and it is likely that their clinical application will become a significant feature in coming years.
A final point to note is that both CR and DR receptors can be used for dual-energy radiography in either of the following configurations:
- Two exposures: where two separate exposures are used in applications where patient movement isn't an issue; and
- Single exposure: where two imaging plates separated by a filter are mounted in a dual-energy cassette to record the low energy image on the anterior plate and the high energy image on the other.
Dual-Energy X-Ray Absorptiometry (DEXA)
[edit | edit source]This technique has its origin in a nuclear medicine procedure where transmission for two gamma ray energies was used to determine bone mineral density. The procedure is referred to as Dual Photon Absorptiometry and typically used the isotope 153Gd, which emits gamma rays at 44 and 100 keV. As a result of limitations in photon flux and practical considerations, the radioactive source has been replaced by an X-ray tube (XRT) in the DEXA technique – just like scanning radioisotope sources have been replaced by XRTs for attenuation correction in SPECT imaging. This method has found widespread clinical application for the assessment and monitoring of osteoporosis and has surpassed the main alternative technique, Quantitative Computed Tomography (QCT) in terms of accuracy, precision and radiation dose.
Two general approaches to generating the appropriate X-ray energies have been developed. In one approach, the kilovoltage and filtration are switched rapidly during image acquisition, e.g. from 70 kVp and 4 mm Al filtration to 140 kVp with an additional 3 mm Cu filter. In the second approach, a single X-ray energy with two different filters is used, e.g. 80 kVp with alternatively no added filtration and a cerium or samarium filter. Cerium has a K absorption edge at 40.4 keV, and samarium at 46.8 keV, and both materials generate a hardened beam compared with the unfiltered spectrum.
The DEXA technique typically involves an X-ray tube and scintillation detector mounted on a C-arm (see the figure below) so that the patient is exposed to a pencil X-ray beam scanning in a rectilinear fashion.
![]() |
![]() |
The pencil beam is used to reduce the detection of scattered radiation and the scintillation detector typically consists of a CdWO4 or NaI(Tl) scintillator coupled to a photomultiplier tube. The filter assembly is used to switch filters and calibration standards into and out of the pencil beam at appropriate intervals. Scan times with this approach are of the order of 2 to 5 minutes, depending on the examination, which are reduced in second generation instruments where a fan X-ray beam and a detector array are used. By rotating the C-arm around the patient during the scan in the second generation devices, a CT image can be formed. The output of the scintillation detector is fed to a computer for dual-energy data processing and image display. A host of body composition parameters can be derived from the image data, e.g. bone mineral density and soft tissue composition.
Notes
This article is a direct transclusion of the Wikibooks article and therefore may not meet the same editing standards as LIMSwiki.